Virtual Tour Of LifeLine (VR-360)
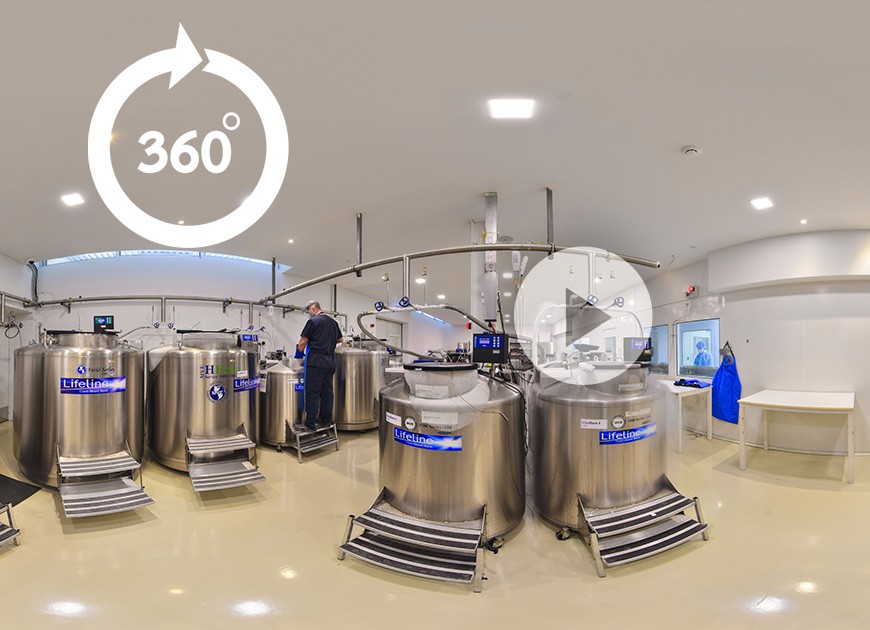
Xinxin Huang 1* , Bin Guo 2* , Maegan Capitano 3* , Hal E. Broxmeyer3
1 Xuhui Hospital and Institutes of Biomedical Sciences, Fudan University, Shanghai, China
2 Department of Pathophysiology, Shanghai Jiao Tong University School of Medicine, Shanghai, China
3 Department of Microbiology and Immunology, Indiana University School of Medicine, Indianapolis, IN, 46202-5181, USA
* Equal contributors
Cord blood (CB) has been used as a viable source of hematopoietic stem cells (HSCs) and hematopoietic progenitor cells (HPCs) in over 35,000 clinical hematopoietic cell transplantation (HCT) efforts to treat the same variety of malignant and non-malignant disorders treated by bone marrow (BM) and mobilized peripheral blood (mPB) using HLA-matched or partially HLA-disparate related or unrelated don
Cord Blood, Hematopoietic Cell Transplantation; Hematopoietic Stem Cells; Hematopoietic Progenitor Cells; Regulation of Hematopoiesis; Collection/Processing of Cord Blood; Influence of Oxygen Tension; Ex-vivo Expansion; Homing
Corresponding author: Hal E. Broxmeyer ( hbroxmey@iupui.edu )
Author roles: Huang X : Conceptualization, Writing – Original Draft Preparation, Writing – Review & Editing; Guo B : Conceptualization, Writing – Original Draft Preparation, Writing – Review & Editing; Capitano M : Conceptualization, Writing – Original Draft Preparation, Writing – Review & Editing; Broxmeyer HE : Conceptualization, Writing – Original Draft Preparation, Writing – Review & Editing
Competing interests: HEB was a member of the Medical Scientific Advisory Board of Cord:Use, a cord blood banking company up until 2018. Cord:Use provided cord blood samples for some of the papers from the Broxmeyer Laboratory but had no input into the use of the cord blood samples provided, for any of the work performed, or for anything in the papers published. HEB started in October 2019 as a member of the Scientific Advisory Board of Elixell, a cell therapy company. Elixell had no input into any of the studies shown or in the writing of this review. The other authors have no competing interests to declare.
Grant information: The studies reported in this review from the Broxmeyer laboratory were supported by the following US Public Health Service grants to Dr. Broxmeyer: R35 HL139599, R01 DK109188, and U54 DK106846. The funders had no role in study design, data collection and analysis, decision to publish, or preparation of the manuscript.
Copyright:© 2019 Huang X et al . This is an open access article distributed under the terms of the Creative Commons Attribution License, which permits unrestricted use, distribution, and reproduction in any medium, provided the original work is properly cited.
How to cite this article: Huang X, Guo B, Capitano M and Broxmeyer HE. Past, present, and future efforts to enhance the efficacy of cord blood hematopoietic cell transplantation [version 1; peer review: 3 approved] F1000Research 2019, 8 (F1000 Faculty Rev):1833 ( https://doi.org/10.12688/f1000research.20002.1 )
First published: 31 Oct 2019, 8 (F1000 Faculty Rev):1833 ( https://doi.org/10.12688/f1000research.20002.1 )
Cord blood (CB) is a clinical source of transplantable hematopoi- etic stem cells (HSCs) and hematopoietic progenitor cells (HPCs) for hematopoietic cell transplantation (HCT) 1-3 . Until the late 1980’s, the cellular source to treat patients with malignant and non-malignant hematopoietic and other disorders by HCT was mainly bone marrow (BM). Mobilized peripheral blood (mPB), where clinicians utilize known agents (e.g. granulocyte colony-stimulating factor 4, 5 and/or HSC/HPC retention signal antagonists such as AMD3100 6-8 ) to release high numbers of HSCs and HPCs into the bloodstream for easy collection, was only in its early stages. In the 1980’s, umbilical CB, usually a discarded material except for routine newborn blood tests, was studied for HSC and HPC biology in a national collaboration 9 . This scientific work was performed at the Indiana University School of Medicine (IUSM). The authors 9 demonstrated that there were likely enough HSCs and HPCs for CB clinical transplantation and tha collected cells could be stored for days at room temperature, shipped by overnight express mail to a distant site, and cryopreserved for future CB HCT. The first proof-of-principle CB storage bank for HLA-matched siblings, set up in the Broxmeyer laboratory 9, 10 , led to the first CB HCT at the Hôpital St. Louis, Paris, in the transplant center directed by Eliane Gluckman as part of an international study 11 . CB cells were collected and sent from a distant obstetric unit to the Broxmeyer laboratory, where they were tested, cryopreserved, and tested again after thawing of a small separate part of the frozen unit before being hand-delivered to Paris for the clinical HCT. On 6 October 1988, the CB unit was infused into a 6-year-old boy with Fanconi anemia who had been first conditioned by a modified regimen specifically for patients with Fanconi anemia that had been previously developed by Dr. Gluckman, utilizing HLA-matched sibling CB from his sister. This first CB HCT 11 was curative for the hematological manifestations of Fanconi anemia; the recipient is alive and well over 31 years later. A total of six more CB HCTs were done in Paris, Baltimore, and Cincinnati using HLA sibling CB cells cryopreserved in the Broxmeyer laboratory to treat Fanconi anemia 10, 12, 13 and juvenile chronic myelogenous leukemia (first CB transplant to treat a leukemia) 14 . Additional information on CB HCT has been reported 1-3,15, 16 and on the functionality of CB HSCs and HPCs 17-30 . Information presented in the original scientific paper was produced years in advance of the clinical transplant, but the scientific 9 and clinical 11 papers were both published in 1989, as we waited until we knew the first clinical CB HCT 11 was successful before submitting the scientific paper 9 . Cryopreserved CB can be stored for at least 23 and a half years 18,31, 32 with little or no loss of HPCs (comparing thawed cells to pre-freeze numbers). CB HCT was extended to partially HLA-disparate and unrelated donors 1-3 . Over 35,000 clinical CB HCT procedures have been performed to date to treat both children and adults with the same malignancies and non-malignancies treated by BM HCT. Advantages of CB HCT are ease of collection and storage of CB without significant risks for the delivering mothers, the ready and quick availability of HLA-typed frozen CB units in public and private CB banks (should such units be rapidly needed for transplantation), and elicitation of relatively low acute and chronic graft versus host disease (GVHD) in recipients after CB HCT, even with unrelated partially HLA- disparate donor cells, compared to that elicited by BM or mPB. Problems include fewer HSCs and HPCs in CB collections than BM or mPB, in part resulting in delayed engraftment of neutrophils, platelets, and immune cells compared to BM and especially with mPB. While not life-threatening, this delay in engraftment with CB prolongs hospital stays, incurring additional health costs 3, 33 .
Efforts to address slower time to donor blood cell recovery have focused on ex vivo (in cell culture) expansion of HSCs (with the idea and possibility that increased numbers of HSCs infused will ameliorate slower time to recovery) or enhancing the homing capacity of HSCs to optimize engraftment. Few such efforts have been tested in the clinical setting 34-40 and only in a few selected transplant centers. This review focuses on enhancing the efficacy of “limited” numbers of HSCs and HPCs in CB collections for CB HCT. A clear distinction must be made between phenotypically recognizable and functional HSCs and HPCs. There are rigorous criteria to phenotypically identify human and mouse HSCs and subsets of HPCs by their cell surface proteins, entailing specific antibodies and flow cytometry. However, phenotype does not necessarily recapitu- late functional status. For functional analysis, one must perform specific engraftment studies in vivo in mice for mouse and human HSCs and colony forming assays in vitro for HPCs 41, 42 . Recent information on collection, ex vivo expansion, and homing of CB HSCs/HPCs for the potential enhancement of CB HCT follows.
Hypoxia is associated with HSC/HPC functions in these cells’ in vivo microenvironment 43 . A means to enhance the efficacy of HCT is through hypoxic collection and processing of HSCs such that the collected cells are never exposed to ambient air oxygen (~21% oxygen) levels 44, 45 . The BM environment, in which HSCs/HPCs reside, has oxygen levels ranging from 1–5%, with some areas possibly being slightly higher or lower depending on proximity to the vasculature 46-49 . Isolating HSCs/HPCs under ambient air (~21% oxygen) exposes these cells to hyperoxic conditions, which within minutes decrease HSC numbers through the differentiation of HSCs to HPCs and not because of HSC cell death 44, 45 . Studies dating from the 1970’s compared culturing of HSCs and HPCs in low (~5% oxygen), in vivo physiological oxygen versus high (~21% oxygen) ambient air oxygen. Culturing human and mouse BM, human CB, and mouse fetal liver at low oxygen in vitro increased numbers of detectable functional HSCs/HPCs 50-56. When cultured in low oxygen (48 mmHg, 6.8% oxygen), clonal growth of granulocyte macrophage progenitors (CFU-GM) from mouse BM was enhanced with increased colony numbers and size compared to a more conventional oxygen environment (135 mmHg, 19% oxygen) 50 . Culturing erythroid progenitors (BFU-E) and more mature erythropoietic precursors (CFU-E) from mouse BM or fetal liver at 5% oxygen increased erythropoietin sensitivity of cells and CFU-E colony numbers 55 . Human low- density CB cells cultured at 5% oxygen had increased CFU-GM, BFU-E, and multipotential progenitors (CFU-GEMM) and were readily expanded ex vivo 56 . Human BM cultured at 5% oxygen had increased CFU-GM numbers 51 . Human BM Lin – CD34 + CD38 – cells (enriched for HSCs) cultured at 1.5% oxygen for 4 days had more functional SCID repopulating cells (an assay for functional human HSCs) than comparable human BM cells cultured at 20% oxygen for 4 days (~5.8-fold increase) or freshly isolated Lin – CD34 + CD38 – cells (~4.2-fold increase) 53 , events associated with stabilization of hypoxia-inducible factor (HIF)-1α, increased surface angiogenic receptors, and VEGF secretion within cultures.
However, in all of these reports, hematopoietic cells were first collected under ambient (~21%) oxygen levels before being placed in culture under lower oxygen and thus the collected cells were already exposed to extra physiological oxygen stress/ shock (EPHOSS) or hyperoxia, which induces the production of mitochondrial reactive oxygen species (ROS), increased HSC differentiation, increased functional HPC numbers and cell cycling, and increased mitochondrial mass/activity. EPHOSS effects are mediated by a p53-cyclophilin D-mitochondria permeability transition pore axis and involve HIF-1α and the hypoxamir miR-21045. Collecting and processing of mouse BM and human CB under low oxygen (3% oxygen, where cells are never exposed to ambient air) resulted in ~two- to five-fold increases in functional HSC numbers (assessed by engraftment in NSG immune-deficient mice) compared to cells collected or processed under ambient air 44, 45. Methods to mimic the effects of low oxygen are being examined. Cyclophilin D inhibitor, cyclosporin A (CSA, used to alleviate GVHD in human HCT), resulted in increased mouse BM and human CB HSC numbers and engraftment capability 45. However, CSA is difficult to work with. It is hard to get into solution and manifests batch-to-batch variations so that each batch needs to be titrated. Combinations of antioxidants and epigenetic enzyme inhibitors within the flush/collection fluids increased numbers of mouse BM HSCs with increased engrafting capacity in a competitive in vivo assay 57, but effects of antioxidants and epigenetic enzyme inhibitors have not yet been verified with human CB cells.
Small molecules, including, but not limited to, diethylaminoben- zaldehyde (DEAB), LG1506, StemRegenin 1 (SR1), UM171, BIO (GSK3β inhibitor), NR-101, trichostatin A (TSA), garcinol (GAR), valproic acid (VPA), copper chelator, tetraethyl- enepentamine, and nicotinamide, are reported agonists for experimental ex vivo expansion of human HSCs and HPCs 58-65. Clinical studies with a few of these small molecules have been reported 35-40. Verification of these clinical studies will take time. SR1 and UM171 are efficient HSC expansion compounds 58,61. SR1, a purine derivative, was identified in a chemical compound screen for candidates promoting ex vivo expansion of human HSCs/HPCs 58. SR1 binds aryl hydrocarbon receptor and antagonizes AhR signaling in CB HSCs/HPCs, but the exact molecular mechanisms remain unclear. SR1 has been tested in a phase I/II clinical trial 40. However, the investigators transplanted both SR1-expanded and -unexpanded CB into patients, so it is too early to determine if SR1-expanded cells contain long-term repopulating HSCs. UM171 promotes ex vivo expansion of long-term repopulating HSCs in experimental models 61, but the clinical trial using UM171 has not yet been published.
Mechanisms behind mouse and human HSC expansion may be different. Neither SR1 nor UM171 stimulates mouse HSC ex vivo expansion 58,61 . Thus, mouse studies to evaluate these molecules are not possible. In contrast, overexpression of HOXB4 or co-culturing of recombinant HOXB4 significantly promoted the expansion of both human CD34 + and mouse HSCs 66, 67 . Activation of OCT4 was found to enhance ex vivo expansion of CB HSCs/HPCs by regulating HOXB4 expression 68 . Angiopoietin-like proteins support mouse and human HSC expansion in culture 69 . Overexpression of MSI2, an oncogene, antagonizes aryl hydrocarbon receptor signaling and expands human HSCs to levels similar to those seen with SR1 70, even though SR1 does not promote mouse HSC expansion 71 .
Readout of HSC expansion is related to the culture systems used, which is one reason why the reproducibility of published research may not be easily confirmed. In our experience, serum-free medium such as SFEM (StemSpan ™ Serum-Free Expansion Medium, Catalog #09650, Stemcell Technologies) or Stemline (Stemline II HSC expansion medium, Catalog #S0192, Sigma-Aldrich) with 100 ng/mL SCF, TPO, and Flt3L can efficiently maintain CD34 + HSC and HPC numbers for 7–10 days. Antagonizing retinoid acid receptor (RAR) or PPAR-gamma (PPAR-G) signaling maintains CD34 + CD38 – stem and progenitor cell populations when CD34 + starting cells are cultured in serum and cytokine-containing RPMI-1640 medium, thus facilitating the expansion or maintenance of HSCs in culture 72, 73. As SFEM medium is effective in maintaining CD34 + CD38 – cell populations, RAR or PPAR-G antagonists do not further enhance the expansion of human HSC production when CD34 + cells are cultured in SFEM medium. PPAR-G expression was repressed when CD34 + cells were cultured in SFEM medium 72, 73. Most recently, a simple HSC ex vivo expansion method was reported by replacing recombinant human serum albumin (HSA) with polyvinyl alcohol (PVA) 74. It was suggested that potential contaminants in recombinant proteins might induce inflammatory responses, thus dampening HSC stemness maintenance. By this minor manipulation, the authors reported a massive 900-fold enhancement in functional HSC numbers after 28-day ex vivo culture 74 . This incredibly efficient expansion system needs to be confirmed by other labs. However, such massive increases in functional HSC numbers may not be needed for enhancement of CB HCT. A few fold increase in these cells may suffice to enhance time to engraftment, although the excess expanded cells can be frozen and stored for additional CB transplants.
N 6 -methyladenosine (m 6 A) modulates the expression of a group of messenger (m) RNAs critical for stem cell fate decisions by modulating their stability 75. Suppressing m 6 A reader, Ythdf2, which promotes targeted decay of mRNA, promotes ex vivo expansion of mouse BM and human CB HSCs 76. Conditional knock-out of mouse Ythdf2 increases functional HSC numbers without changing lineage differentiation and without apparent manifestation of hematological malignancies. Knockdown of human YTHDF2 resulted in a 10-fold increase in cytokine- mediated ex vivo expansion of human CB HSCs, a 5-fold increase in HPCs, and a greater than 8-fold increase in serial trans- plantation 76. This was associated with enrichment in mRNAs encoding transcription factors in HSCs previously shown to be critical for stem cell renewal. This procedure thus targets multiple effectors rather than one 76, 77.
Epigenetic reprogramming using VPA has been utilized to experimentally expand human CB HSCs ex vivo 59. This required the coordination of cellular reprogramming with remodeling of mitochondria and activation of p53 that apparently limits ROS levels 78, which induce HSC differentiation 44, 45 . DEK, a nuclear heterochromatin remodeling agent, which can be secreted from the cell and act as a cytokine that manifests its effects through the chemokine receptor CXCR2 (the only known non-chemokine to bind CXCR2), enhances cytokine-mediated ex vivo expansion of human CB and mouse BM HSCs and HPCs 79.
One challenge for ex vivo expansion is the lack of markers labeling functional HSCs during and after ex vivo culture. Some signaling pathways might stimulate the expansion of CD34 + cells, most of which are progenitors. Markers including CD90 or CD49f have often been used to isolate HSCs from fresh human CB and BM samples. However, CD34 + CD90 + CD49f + phenotypic HSCs do not necessarily reflect functional HSCs, especially under stress conditions such as ex vivo expansion 41. The only currently available way to confirm the expansion of human HSC numbers is through transplantation using sublethally irradiated immune-deficient mice. A logistical problem is that ex vivo clinical studies will likely have to be performed in very select centers with expertise for these procedures. Also, the economics associated with ex vivo expansion must be taken into account, as it will likely add significant additional costs to the clinical HCT procedure. While it does not appear that ex vivo expansion procedures have damaged HSCs or caused pre-leukemia/leukemia, anytime HSCs are manipulated ex vivo, there is potential for long-term detrimental effects, which may involve gene expression pattern changes and epigenetic modifications that might result in long-term counter-productive outcomes.
During ex vivo expansion, we must also keep in mind the different physical characteristics of the in vivo HSC BM niche that help maintain HSC homeostasis (e.g. interactions that HSCs have with the other cells within their BM niche and lower oxygen concentrations within the BM). Taking these factors into account, CB HSCs/HPCs have been expanded in the presence of mesenchymal stem/stromal cells and have been proven to be safe in a clinical study 36. In addition, hypoxia culturing (5% oxygen) after cells were collected in ambient air potentiated ex vivo expansion of CB HSCs/HPCs 80.
Although ex vivo expansion is a promising means to overcome limited numbers of CB HSCs collected for transplantation, there is still much work to be done in this area. More mechanistic insight is required regarding the regulation of HSC stemness.
After infusion into peripheral blood, HSCs home to the BM microenvironment by sensing chemical gradients of chemoattractants 81. The BM microenvironment provides a unique matrix bedding and conducive signaling environment supporting long-term engraftment and balances HSC proliferation and differentiation 82, 83. HSC homing is crucial for successful clinic outcomes 84.
Directing HSC migration and homing from the peripheral circulation to the BM involves interactions between chemokine ligand CXCL12/stromal cell-derived factor (SDF)-1 and its receptor CXCR4 85, 86. CXCL12 is highly expressed by BM stromal cells padding the stem cell niche. Gradients of CXCL12 provide directional cues and orchestrate HSC migration towards the BM. CXCR4 is a seven-transmembrane G-protein- coupled chemokine receptor expressed on the surface of HSCs. Knockouts of CXCL12 or CXCR4 result in severe hematopoietic defects 87-89 . Sphingosine-1-phosphate (S1P) and ceramide-1-phosphate (C1P) also provide homing gradients guiding HSCs to BM niches 90-92. Strategies to enhance HSC homing are classified into three categories: regulation from the cell membrane, regulation in the cytoplasm, and regulation in the nucleus.
The cell membrane is a semi-permeable membrane separating the cell from the external environment. The cell membrane consists of phospholipid bilayers and many membrane-associated proteins, while lipids are fundamental structural elements, and proteins are responsible for performing specific membrane functions 93, 94. Lipid rafts are special membrane domains rich in glycosphingolipids and cholesterol and have been implicated in regulating membrane signaling 95, 96. Incorporation of CXCR4 into lipid rafts facilitates the sensing of CXCL12 gradients, enhancing homing and engraftment of HSCs 97, 98. Short-term (4-hour) mild heating (39°C) led to elevated membrane lipid raft formation, resulting in increased CXCR4 aggregation and co-localization with lipid rafts, and promoted human CB HSC homing and engraftment in an NSG mouse transplantation model 99. One report showed a beneficial effect of dimethyl sulfoxide (DMSO) treatment on HSC homing, possibly because of lower internalization of the surface CXCR4 receptor 100.
Another HSC homing regulator found on the cell membrane is dipeptidyl peptidase 4 (DPP4). DPP4, also referred to as cell surface CD26, a 110 kDa serine protease. It cleaves penultimate alanine or proline amino acids at the N-terminus of target sub- strates including cytokines and chemokines 101, 102. DPP4 is widely expressed in tissues, e.g. liver, spleen, lung, and BM, as a membrane-bound form and is also found in serum in soluble form. DPP4 is expressed on the surface of HSCs and HPCs, as well as on T lymphocytes; it is an important regulator of HSC and T cell function 101-103 and modulates HSC homing at least in part by modifying CXCL12 104, 105. DPP4 generates a truncated form of CXCL12, which is no longer chemotactic but is able to block chemotaxis of full-length CXCL12 104. Blocking enzymatic activity of DPP4 increases levels of non-truncated CXCL12, enhancing HSC homing and engraftment of human CB CD34 + cells or mouse BM cells 105, 106. Sitagliptin, an FDA-approved orally active inhibitor of DPP4, has been used to enhance the engraftment of single CB transplantation in patients with leukemia and lymphoma 107-109. Since it was subsequently realized that DPP4 truncated a number of other hematopoietic-regulating cytokines 110, the time to engraftment may have been further decreased had sitagliptin been given over more days.
Prostaglandin E2 (PGE 2 ) is an important mediator of physi- ological and pathological systems 111, 112. Pulse treatment of human and murine HSCs with PGE 2 results in enhanced HSC homing and engraftment, mediated through the upregulation of surface CXCR4 levels 22. A clinical trial evaluating the effects of PGE 2 in CB HCT has elicited promising results, with apparently faster neutrophil recovery and long-term dominance of the PGE 2 - treated CB unit 34, but this study was done using PGE 2 -treated and -untreated CB. The long-term engrafting capability of the PGE 2 -treated cells is unknown. PGE 2 has four specific G-protein-coupled receptors on the cell membrane, EP1–EP4 113. EP2 and EP4 were involved in the upregulation of CXCR4 and CXCL12 expression and promoted HSC migration towards CXCL12 114. It may be practical to develop better EP2 and EP4 agonists to further enhance HSC homing and engraftment. In an animal model, combining PGE 2 treatment of donor cells and in vivo DPP4 inhibitor demonstrated additive effects on enhancing mouse BM engraftment into lethally irradiated mice 115, suggesting potential enhancement in efficacy by combining two different treatment modalities.
Calcium-sensing receptor (CaR) is a cell membrane G-protein- coupled receptor mediating cell responses to extracellular calcium 116. CaR knockout HSCs are defective in adherence to the BM microenvironment and fail to engraft after trans- plantation 117. Treatment of murine HSCs with a CaR agonist, cinacalcet, led to enhanced HSC homing and engraftment, effects mediated through intracellular CXCR4 signaling 117. CXCR4 mRNA and surface expression remained unaltered, so cinacalcet may stimulate enhanced CXCR4 signaling in an unconventional manner.
During the homing process, the first early step is considered to be HSCs rolling on P-selectins and E-selectins of endothelial cells in BM 30. P-selectins and E-selectins are C-type lectins whose ligands must be properly α1,3-fucosylated to form mature glycan determinants. Increasing the levels of cell surface fucosylation has been shown to enhance the engraftment of CB cells in immunodeficient mice 30, 118, 119. Furthermore, CB units were treated with guanosine diphosphate fucose and fucosyl- transferase-VI to enhance cell surface fucosylation in a clinical trial, and the results showed improved engraftment efficiency of fucosylated cells 27.
Heme oxygenase 1 (HO-1), an endoplasmic reticulum (ER)-anchored enzyme, plays important roles in anti-oxidative and inflammatory processes 120. HO-1 acts as a negative regulator of HSC homing. HO-1 knockout HSCs have enhanced migration towards CXCL12 and S1P gradients 121. Transient treatment with HO-1 inhibitor (SnPP) increased chemotaxis and homing of HSCs/HPCs 121.
The glucocorticoid receptor (GR) is an evolutionarily conserved nuclear receptor to which glucocorticoids bind 122, 123. Upon ligand binding, GR is transported into the nucleus and functions as a transcriptional factor to activate downstream gene transcription, regulating numerous physiological processes. Glucocorticoid treatment of human CB HSCs significantly elevated surface CXCR4 expression and increased chemotaxis towards CXCL12, HSC homing, and engraftment in NSG mice 123. Activated GR transfers into the nucleus and binds to glucocorticoid response elements in the CXCR4 promoter in human CB HSCs, followed by recruitment of SRC1/p300 histone acetyltransferase complex. This promotes histone H4K5 and H4K16 acetylation in the CXCR4 promoter region, leading to upregulation of CXCR4 transcription. Knockdown of SRC1 or p300 suppresses the effects of activated GR on CXCR4 surface expression, while inhibition of p300 by a small molecule inhibitor, C646, blocks the enhanced homing effects of activated GR 72, suggesting that activated GR depends on histone acetylation to promote HSC homing.
Histone deacetylases (HDACs) are crucial modulators in regu- lating histone acetylation levels 124, 125. HDACs remove acetyl groups from lysines of target proteins and play important roles in physiological processes 124. The treatment of human CB HSCs with HDAC inhibitors substantially increased surface CXCR4 expression, improved chemotaxis towards CXCL12, and enhanced HSC homing and engraftment 126. There are 18 HDAC enzymes in mammals, grouped into five subfamilies based on sequence similarity (class I, IIa, IIb, III, and IV) 127. HDAC5 is the one HDAC specifically involved in the regulation of CXCR4 expression and HSC homing 128. HDAC5 inhibition increased acetylation levels of histones at the CXCR4 promoter region as well as p65 acetylation levels in the nucleus. NF-κB subunit p65 is a crucial transcription factor regulating CXCR4 expres- sion. The acetylation of p65 enhances its DNA-binding activity and promotes target gene transcription 127-130. Blocking NF-κB signaling suppressed the effects of HDAC5 inhibition on CXCR4 upregulation and enhanced HSC homing 126, 128, indicating essential roles for NF-κB signaling in regulating HSC homing and demonstrating a previously unknown negative regulation of HSC homing by HDAC5.
HIF-1α, a DNA-binding transcriptional factor, mediates cel- lular responses to hypoxia 43, 129. HIF-1α is important during animal development and for energy metabolism. The BM microenvironment, where HSCs reside, is hypoxic 43. HIF-1α is stabilized in HSCs and regulates HSC activity/quiescence 131, 132. Pharmacological increases in HIF-1α promote HSC hom- ing and engraftment also by upregulating surface CXCR4 expression 133. CXCR4 expression upregulation results from HIF-1α binding with hypoxia response elements, located at –1.3 kb from the transcription start site of the CXCR4 promoter region. Caffeic acid phenethyl ester (CAPE) treat- ment promotes HSC homing and engraftment by inducing the expression of HIF-1α. CAPE administration upregulates HIF-1α protein levels and CXCL12 in BM endothelial cells and inhibition of HIF-1α by PX-478 suppresses CAPE-mediated enhanced HSC homing, further supporting the notion that HIF-1α is important during HSC homing and engraftment.
The above-mentioned approaches for homing range from the cell membrane (lipid rafts, DPP4, EP2 and EP4, and CaR) to the cytoplasm (HO-1) and inside the nucleus (GR, HDAC5, and HIF-1α). Which procedure would be the best to be tested in a clinical setting needs to be determined. Perhaps combinations of approaches can further increase the homing and engraftment of HSCs. It may be that short-term treatment of donor CB units for about 16 hours may provide significant enhancement for engraftment in the setting of CB HCT, possibly negating the necessity for ex vivo expansion efforts. Alternatively, it may be that the CB cells do not have to be pretreated ex vivo prior to infusion into the patient but rather that the cells can be infused into the patient who is then given the reagents in vivo to enhance the homing/engrafting capability of the infused cells. It is also possible that ex vivo expanded HSCs may better engraft if it turns out that homing of expanded HSCs is suboptimal and can be enhanced.
Enhancing CB HCT efficacy will not only reduce the time of donor cell recovery but also make it possible to use more banked CB units that contain fewer HSCs/HPCs. There are a number of new ways to potentially enhance the efficacy of CB HCT 134. However, most are laboratory efforts. How to get these new methods into clinical trials is a problem that needs to be solved. We believe that simpler is always better. The simpler the procedure, the more likely that it will be clinically translated. There are just not enough clinical CB HCTs available to set up phase I/II clinical trials to test these new procedures. Most investigators doing such trials are “wed” to their personal favorite procedure. If, in the future, we can deal with this problem and find means for additional clinical efforts, it is possible that several new procedures can be used together 134. This, however, adds additional logistical problems versus the use of one procedure. Clinical trials are costly, and it is not clear where the money to pursue such trials will come from, or even if they can be supported at all, since current trials are funded by companies to test their own products. A gathering of interested scientists and clinical investigators who can think-tank this problem is desperately needed and strongly encouraged.
F1000 Recommendation | PubMed Abstract | Publisher Full Text | Free Full Text
1. Ballen KK, Gluckman E, Broxmeyer HE: Umbilical cord blood transplantation: the first 25 years and beyond. Blood. 2013; 122(4): 491–8.
2. Broxmeyer HE, Farag SS, Rocha V: Cord blood hematopoietic cell transplantation. In: Forman SJ, Negrin RS, Antin JH, Appelbaum FR, editors. Thomas’ Hematopoietic Cell Transplantation. 5th ed. Oxford, England: John Wiley & Sons, Ltd; 2016; 437–55.
3. Mayani H, Wagner JE, Broxmeyer HE: Cord blood research, banking, and transplantation: achievements, challenges, and perspectives. Bone Marrow Transplant. 2019.
4. de Kruijf EFM, Fibbe WE, van Pel M: Cytokine-induced hematopoietic stem and progenitor cell mobilization: unraveling interactions between stem cells and their niche. Ann N Y Acad Sci. 2019.
5. Pelus LM, Broxmeyer HE: Peripheral blood stem cell mobilization; a look ahead. Curr Stem Cell Rep. 2018; 4(4): 273–81.
6. Broxmeyer HE, Orschell CM, Clapp DW, et al.: Rapid mobilization of murine and human hematopoietic stem and progenitor cells with AMD3100, a CXCR4 antagonist. J Exp Med. 2005; 201(8): 1307–18.
7. Liles WC, Broxmeyer HE, Rodger E, et al.: Mobilization of hematopoietic progenitor cells in healthy volunteers by AMD3100, a CXCR4 antagonist. Blood. 2003; 102(8): 2728–30.
8. Liles WC, Rodger E, Broxmeyer HE, et al.: Augmented mobilization and collection of CD34+ hematopoietic cells from normal human volunteers stimulated with granulocyte-colony-stimulating factor by single-dose administration of AMD3100, a CXCR4 antagonist. Transfusion. 2005; 45(3): 295–300.
9. Broxmeyer HE, Douglas GW, Hangoc G, et al.: Human umbilical cord blood as a potential source of transplantable hematopoietic stem/progenitor cells. Proc Natl Acad Sci U S A. 1989; 86(10): 3828–32.
10. Broxmeyer HE, Gluckman E, Auerbach A, et al.: Human umbilical cord blood: a clinically useful source of transplantable hematopoietic stem/progenitor cells. Int J Cell Cloning. 1990; 8 Suppl 1: 76–89; discussion 89–91.
11. Gluckman E, Broxmeyer HA, Auerbach AD, et al.: Hematopoietic reconstitution in a patient with Fanconi’s anemia by means of umbilical-cord blood from an HLA-identical sibling. N Engl J Med. 1989; 321(17): 1174–8.
12. Broxmeyer HE, Kurtzberg J, Gluckman E, et al.: Umbilical cord blood hematopoietic stem and repopulating cells in human clinical transplantation. Blood Cells. 1991; 17(2): 313–29.
13. Kohli-Kumar M, Shahidi NT, Broxmeyer HE, et al.: Haemopoietic stem/progenitor cell transplant in Fanconi anaemia using HLA-matched sibling umbilical cord blood cells. Br J Haematol. 1993; 85(2): 419–22.
14. Wagner JE, Broxmeyer HE, Byrd RL, et al.: Transplantation of umbilical cord blood after myeloablative therapy: analysis of engraftment. Blood. 1992; 79(7): 1874–81.
15. Broxmeyer HE: The history of cord blood transplantation/biology & perspective for future efforts to enhance the field. Cell Gene Therapy Insights. 2017; 3(7): 521–30.
16. Wagner JE, Kernan NA, Steinbuch M, et al.: Allogeneic sibling umbilical-cordblood transplantation in children with malignant and non-malignant disease. Lancet. 1995; 346(8969): 214–9.
17. Bock TA, Orlic D, Dunbar CE, et al.: Improved engraftment of human hematopoietic cells in severe combined immunodeficient (SCID) mice carrying human cytokine transgenes. J Exp Med. 1995; 182(6): 2037–43.
18. Broxmeyer HE, Cooper S: High-efficiency recovery of immature haematopoietic progenitor cells with extensive proliferative capacity from human cord blood cryopreserved for 10 years. Clin Exp Immunol. 1997; 107 Suppl 1: 45–53.
19. Broxmeyer HE, Hangoc G, Cooper S, et al.: Growth characteristics and expansion of human umbilical cord blood and estimation of its potential for transplantation in adults. Proc Natl Acad Sci U S A. 1992; 89(9): 4109–13.
20. Carow CE, Hangoc G, Broxmeyer HE: Human multipotential progenitor cells (CFU-GEMM) have extensive replating capacity for secondary CFU-GEMM: an effect enhanced by cord blood plasma. Blood. 1993; 81(4): 942–9.
21. Csaszar E, Kirouac DC, Yu M, et al.: Rapid expansion of human hematopoietic stem cells by automated control of inhibitory feedback signaling. Cell Stem Cell. 2012; 10(2): 218–29.
22. Hoggatt J, Singh P, Sampath J, et al.: Prostaglandin E2 enhances hematopoietic stem cell homing, survival, and proliferation. Blood. 2009; 113(22): 5444–55.
23. Lu L, Xiao M, Shen RN, et al.: Enrichment, characterization, and responsiveness of single primitive CD34 human umbilical cord blood hematopoietic progenitors with high proliferative and replating potential. Blood. 1993; 81(1): 41–8.
24. Mayani H, Lansdorp PM: Biology of human umbilical cord blood-derived hematopoietic stem/progenitor cells. Stem Cells. 1998; 16(3): 153–65.
25. Peled T, Mandel J, Goudsmid RN, et al.: Pre-clinical development of cord blood-derived progenitor cell graft expanded ex vivo with cytokines and the polyamine copper chelator tetraethylenepentamine. Cytotherapy. 2004; 6(4): 344–55.
26. Piacibello W, Sanavio F, Garetto L, et al.: Extensive amplification and selfrenewal of human primitive hematopoietic stem cells from cord blood. Blood. 1997; 89(8): 2644–53.
27. Popat U, Mehta RS, Rezvani K, et al.: Enforced fucosylation of cord blood hematopoietic cells accelerates neutrophil and platelet engraftment after transplantation. Blood. 2015; 125(19): 2885–92.
28. Vormoor J, Lapidot T, Pflumio F, et al.: Immature human cord blood progenitors engraft and proliferate to high levels in severe combined immunodeficient mice. Blood. 1994; 83(9): 2489–97.
29. Vormoor J, Lapidot T, Pflumio F, et al.: SCID mice as an in vivo model of human cord blood hematopoiesis. Blood Cells. 1994; 20(2–3): 316–20:discussion 320–2.
30. Xia L, McDaniel JM, Yago T, et al.: Surface fucosylation of human cord blood cells augments binding to P-selectin and E-selectin and enhances engraftment in bone marrow. Blood. 2004; 104(10): 3091–6.
31. Broxmeyer HE, Lee MR, Hangoc G, et al.: Hematopoietic stem/progenitor cells, generation of induced pluripotent stem cells, and isolation of endothelial progenitors from 21- to 23.5-year cryopreserved cord blood. Blood. 2011; 117(18): 4773–7.
32. Broxmeyer HE, Srour EF, Hangoc G, et al.: High-efficiency recovery of functional hematopoietic progenitor and stem cells from human cord blood cryopreserved for 15 years. Proc Natl Acad Sci U S A. 2003; 100(2): 645–50.
33. Broxmeyer HE, Farag S: Background and future considerations for human cord blood hematopoietic cell transplantation, including economic concerns. Stem Cells Dev. 2013; 22 Suppl 1: 103–10.
34. Cutler C, Multani P, Robbins D, et al.: Prostaglandin-modulated umbilical cord blood hematopoietic stem cell transplantation. Blood. 2013; 122(17): 3074–81.
35. de Lima M, McMannis J, Gee A, et al.: Transplantation of ex vivo expanded cord blood cells using the copper chelator tetraethylenepentamine: a phase I/II clinical trial. Bone Marrow Transplant. 2008; 41(9): 771–8.
36. de Lima M, McNiece I, Robinson SN, et al.: Cord-blood engraftment with ex vivo mesenchymal-cell coculture. N Engl J Med. 2012; 367(24): 2305–15.
37. Delaney C, Heimfeld S, Brashem-Stein C, et al.: Notch-mediated expansion of human cord blood progenitor cells capable of rapid myeloid reconstitution. Nat Med. 2010; 16(2): 232–6.
38. Horwitz ME, Chao NJ, Rizzieri DA, et al.: Umbilical cord blood expansion with nicotinamide provides long-term multilineage engraftment. J Clin Invest. 2014; 124(7): 3121–8.
39. Horwitz ME, Wease S, Blackwell B, et al.: Phase I/II Study of Stem-Cell Transplantation Using a Single Cord Blood Unit Expanded Ex Vivo With Nicotinamide. J Clin Oncol. 2019; 37(5): 367–74.
40. Wagner JE Jr, Brunstein CG, Boitano AE, et al.: Phase I/II Trial of StemRegenin-1 Expanded Umbilical Cord Blood Hematopoietic Stem Cells Supports Testing as a Stand-Alone Graft. Cell Stem Cell. 2016; 18(1): 144–55.
41. Chen Y, Yao C, Teng Y, et al.: Phorbol ester induced ex vivo expansion of rigorously-defined phenotypic but not functional human cord blood hematopoietic stem cells: a cautionary tale demonstrating that phenotype does not always recapitulate stem cell function. Leukemia. 2019.
42. Dorrell C, Gan OI, Pereira DS, et al.: Expansion of human cord blood CD34(+)CD38(-) cells in ex vivo culture during retroviral transduction without a corresponding increase in SCID repopulating cell (SRC) frequency: dissociation of SRC phenotype and function. Blood. 2000; 95(1): 102–10.
43. Huang X, Trinh T, Aljoufi A, et al.: Hypoxia Signaling Pathway in Stem Cell Regulation: Good and Evil. Curr Stem Cell Rep. 2018; 4(2): 149–57.
44. Broxmeyer HE, O'Leary HA, Huang X, et al.: The importance of hypoxia and extra physiologic oxygen shock/stress for collection and processing of stem and progenitor cells to understand true physiology/pathology of these cells ex vivo. Curr Opin Hematol. 2015; 22(4): 273–8.
45. Mantel CR, O'Leary HA, Chitteti BR, et al.: Enhancing Hematopoietic Stem Cell Transplantation Efficacy by Mitigating Oxygen Shock. Cell. 2015; 161(7): 1553–65.
46. Morrison SJ, Scadden DT: The bone marrow niche for haematopoietic stem cells. Nature. 2014; 505(7483): 327–34.
47. Nombela-Arrieta C, Pivarnik G, Winkel B, et al.: Quantitative imaging of haematopoietic stem and progenitor cell localization and hypoxic status in the bone marrow microenvironment. Nat Cell Biol. 2013; 15(5): 533–43.
48. Sjöstedt S, Rooth G, Caligara F: The Oxygen Tension of the Blood in the Umbilical Cord and the Intervillous Space. Arch Dis Child. 1960; 35(184): 529–33.
49. Spencer JA, Ferraro F, Roussakis E, et al.: Direct measurement of local oxygen concentration in the bone marrow of live animals. Nature. 2014; 508(7495): 269–73.
50. Bradley TR, Hodgson GS, Rosendaal M: The effect of oxygen tension on haemopoietic and fibroblast cell proliferation in vitro. J Cell Physiol. 1978; 97(3 Pt 2 Suppl 1): 517–22.
51. Broxmeyer HE, Cooper S, Gabig T: The effects of oxidizing species derived from molecular oxygen on the proliferation in vitro of human granulocytemacrophage progenitor cells. Ann N Y Acad Sci. 1989; 554: 177–84.
52. Broxmeyer HE, Cooper S, Rubin BY, et al.: The synergistic influence of human interferon-gamma and interferon-alpha on suppression of hematopoietic progenitor cells is additive with the enhanced sensitivity of these cells to inhibition by interferons at low oxygen tension in vitro. J Immunol. 1985; 135(4): 2502–6.
53. Danet GH, Pan Y, Luongo JL, et al.: Expansion of human SCID-repopulating cells under hypoxic conditions. J Clin Invest. 2003; 112(1): 126–35.
54. Lu L, Broxmeyer HE: Comparative influences of phytohemagglutinin-stimulated leukocyte conditioned medium, hemin, prostaglandin E, and low oxygen tension on colony formation by erythroid progenitor cells in normal human bone marrow. Exp Hematol. 1985; 13(10): 989–93.
55. Rich IN, Kubanek B: The effect of reduced oxygen tension on colony formation of erythropoietic cells in vitro. Br J Haematol. 1982; 52(4): 579–88.
56. Smith S, Broxmeyer HE: The influence of oxygen tension on the long-term growth in vitro of haematopoietic progenitor cells from human cord blood. Br J Haematol. 1986; 63(1): 29–34.
57. Cai Q, Capitano M, Huang X, et al.: Combinations of antioxidants and/or of epigenetic enzyme inhibitors allow for enhanced collection of mouse bone marrow hematopoietic stem cells in ambient air. Blood Cells Mol Dis. 2018; 71: 23–8.
58. Boitano AE, Wang J, Romeo R, et al.: Aryl hydrocarbon receptor antagonists promote the expansion of human hematopoietic stem cells. Science. 2010; 329(5997): 1345–8.
59. Chaurasia P, Gajzer DC, Schaniel C, et al.: Epigenetic reprogramming induces the expansion of cord blood stem cells. J Clin Invest. 2014; 124(6): 2378–95.
60. Chute JP, Muramoto GG, Whitesides J, et al.: Inhibition of aldehyde dehydrogenase and retinoid signaling induces the expansion of human hematopoietic stem cells. Proc Natl Acad Sci U S A. 2006; 103(31): 11707–12.
61. Fares I, Chagraoui J, Gareau Y, et al.: Cord blood expansion. Pyrimidoindole derivatives are agonists of human hematopoietic stem cell self-renewal. Science. 2014; 345(6203): 1509–12.
62. Ko KH, Holmes T, Palladinetti P, et al.: GSK-3β inhibition promotes engraftment of ex vivo-expanded hematopoietic stem cells and modulates gene expression. Stem Cells. 2011; 29(1): 108–18.
63. Nishino T, Miyaji K, Ishiwata N, et al.: Ex vivo expansion of human hematopoietic stem cells by a small-molecule agonist of c-MPL. Exp Hematol. 2009; 37(11): 1364–1377.
64. Nishino T, Wang C, Mochizuki-Kashio M, et al.: Ex vivo expansion of human hematopoietic stem cells by garcinol, a potent inhibitor of histone acetyltransferase. PLoS One. 2011; 6(9): e24298.
65. Safi R, Muramoto GG, Salter AB, et al.: Pharmacological manipulation of the RAR/RXR signaling pathway maintains the repopulating capacity of hematopoietic stem cells in culture. Mol Endocrinol. 2009; 23(2): 188–201.
66. Antonchuk J, Sauvageau G, Humphries RK: HOXB4-induced expansion of adult hematopoietic stem cells ex vivo. Cell. 2002; 109(1): 39–45.
67. Krosl J, Austin P, Beslu N, et al.: In vitro expansion of hematopoietic stem cells by recombinant TAT-HOXB4 protein. Nat Med. 2003; 9(11): 1428–32.
68. Huang X, Lee MR, Cooper S, et al.: Activation of OCT4 enhances ex vivo expansion of human cord blood hematopoietic stem and progenitor cells by regulating HOXB4 expression. Leukemia. 2016; 30(1): 144–53.
69. Zhang CC, Kaba M, Ge G, et al.: Angiopoietin-like proteins stimulate ex vivo expansion of hematopoietic stem cells. Nat Med. 2006; 12(2): 240–5.
70. Rentas S, Holzapfel N, Belew MS, et al.: Musashi-2 attenuates AHR signalling to expand human haematopoietic stem cells. Nature. 2016; 532(7600): 508–11.
71. Kharas MG, Lengner CJ, Al-Shahrour F, et al.: Musashi-2 regulates normal hematopoiesis and promotes aggressive myeloid leukemia. Nat Med. 2010; 16(10): 903–8.
72. Guo B, Huang X, Broxmeyer HE: Enhancing human cord blood hematopoietic stem cell engraftment by targeting nuclear hormone receptors. Curr Opin Hematol. 2018; 25(4): 245–52.
73. Guo B, Huang X, Lee MR, et al.: Antagonism of PPAR-γ signaling expands human hematopoietic stem and progenitor cells by enhancing glycolysis. Nat Med. 2018; 24(3): 360–7.
74. Wilkinson AC, Ishida R, Kikuchi M, et al.: Long-term ex vivo haematopoieticstem-cell expansion allows nonconditioned transplantation. Nature. 2019; 571(7763): 117–21.
75. Guo M, Liu X, Zheng X, et al.: m6 A RNA Modification Determines Cell Fate by Regulating mRNA Degradation. Cell Reprogram. 2017; 19(4): 225–31.
76. Li Z, Qian P, Shao W, et al.: Suppression of m6 A reader Ythdf2 promotes hematopoietic stem cell expansion. Cell Res. 2018; 28(9): 904–17.
77. Huang X, Broxmeyer HE: m6 A reader suppression bolsters HSC expansion. Cell Res. 2018; 28(9): 875–6.
78. Papa L, Zimran E, Djedaini M, et al.: Ex vivo human HSC expansion requires coordination of cellular reprogramming with mitochondrial remodeling and p53activation. Blood Adv. 2018; 2(20): 2766–79.
79. Capitano ML, Mor-Vaknin N, Saha AK, et al.: Secreted nuclear protein DEK regulates hematopoiesis through CXCR2 signaling. J Clin Invest. 2019; 129(6): 2555–70.
80. Roy S, Tripathy M, Mathur N, et al.: Hypoxia improves expansion potential of human cord blood-derived hematopoietic stem cells and marrow repopulation efficiency. Eur J Haematol. 2012; 88(5): 396–405.
81. Lapidot T, Dar A, Kollet O: How do stem cells find their way home? Blood. 2005; 106(6): 1901–10.
82. Crane GM, Jeffery E, Morrison SJ: Adult haematopoietic stem cell niches. Nat Rev Immunol. 2017; 17(9): 573–90.
83. Yu VWC, Scadden DT: Heterogeneity of the bone marrow niche. Curr Opin Hematol. 2016; 23(4): 331–8.
84. Huang X, Broxmeyer HE: Progress towards improving homing and engraftment of hematopoietic stem cells for clinical transplantation. Curr Opin Hematol. 2019; 26(4): 266–72.
85. Kim CH, Broxmeyer HE: In vitro behavior of hematopoietic progenitor cells under the influence of chemoattractants: Stromal cell-derived factor-1, steel factor, and the bone marrow environment. Blood. 1998; 91(1): 100–10.
86. Nagasawa T: CXCL12/SDF-1 and CXCR4. Front Immunol. 2015; 6: 301.
87. Ma Q, Jones D, Borghesani PR, et al.: Impaired B-lymphopoiesis, myelopoiesis, and derailed cerebellar neuron migration in CXCR4- and SDF-1-deficient mice. Proc Natl Acad Sci U S A. 1998; 95(16): 9448–53.
88. Nagasawa T, Hirota S, Tachibana K, et al.: Defects of B-cell lymphopoiesis and bone-marrow myelopoiesis in mice lacking the CXC chemokine PBSF/SDF-1. Nature. 1996; 382(6592): 635–8.
89. Zou YR, Kottmann AH, Kuroda M, et al.: Function of the chemokine receptor CXCR4 in haematopoiesis and in cerebellar development. Nature. 1998; 393(6685): 595–9.
90. Golan K, Vagima Y, Ludin A, et al.: S1P promotes murine progenitor cell egress and mobilization via S1P1-mediated ROS signaling and SDF-1 release. Blood. 2012; 119(11): 2478–88.
91. Juarez JG, Harun N, Thien M, et al.: Sphingosine-1-phosphate facilitates trafficking of hematopoietic stem cells and their mobilization by CXCR4 antagonists in mice. Blood. 2012; 119(3): 707–16.
92. Ratajczak MZ, Lee H, Wysoczynski M, et al.: Novel insight into stem cell mobilization-plasma sphingosine-1-phosphate is a major chemoattractant that directs the egress of hematopoietic stem progenitor cells from the bone marrow and its level in peripheral blood increases during mobilization due to activation of complement cascade/membrane attack complex. Leukemia. 2010; 24(5): 976–85.
93. Goñi FM: The basic structure and dynamics of cell membranes: an update of the Singer-Nicolson model. Biochim Biophys Acta. 2014; 1838(6): 1467–76.
94. Nicolson GL: The Fluid-Mosaic Model of Membrane Structure: still relevant to understanding the structure, function and dynamics of biological membranes after more than 40 years. Biochim Biophys Acta. 2014; 1838(6): 1451–66.
95. Ando J, Kinoshita M, Cui J, et al.: Sphingomyelin distribution in lipid rafts of artificial monolayer membranes visualized by Raman microscopy. Proc Natl Acad Sci U S A. 2015; 112(15): 4558–63.
96. Simons K, Ehehalt R: Cholesterol, lipid rafts, and disease. J Clin Invest. 2002; 110(5): 597–603.
97. Ratajczak MZ, Adamiak M: Membrane lipid rafts, master regulators of hematopoietic stem cell retention in bone marrow and their trafficking. Leukemia. 2015; 29(7): 1452–7.
98. Wysoczynski M, Reca R, Ratajczak J, et al.: Incorporation of CXCR4 into membrane lipid rafts primes homing-related responses of hematopoietic stem/progenitor cells to an SDF-1 gradient. Blood. 2005; 105(1): 40–8.
99. Capitano ML, Hangoc G, Cooper S, et al.: Mild Heat Treatment Primes Human CD34+ Cord Blood Cells for Migration Toward SDF-1α and Enhances Engraftment in an NSG Mouse Model. Stem Cells. 2015; 33(6): 1975–84.
100. Jarocha D, Zuba-Surma E, Majka M: Dimethyl Sulfoxide (DMSO) Increases Percentage of CXCR4+ Hematopoietic Stem/Progenitor Cells, Their Responsiveness to an SDF-1 Gradient, Homing Capacities, and Survival. Cell Transplant. 2016; 25(7): 1247–57.
101. O'Leary H, Ou X, Broxmeyer HE: The role of dipeptidyl peptidase 4 in hematopoiesis and transplantation. Curr Opin Hematol. 2013; 20(4): 314–9.
102. Ou X, O'Leary HA, Broxmeyer HE: Implications of DPP4 modification of proteins that regulate stem/progenitor and more mature cell types. Blood. 2013; 122(2): 161–9.
103. Ohnuma K, Takahashi N, Yamochi T, et al.: Role of CD26/dipeptidyl peptidase IV in human T cell activation and function. Front Biosci. 2008; 13: 2299–310.
104. Christopherson KW, Hangoc G, Broxmeyer HE: Cell surface peptidase CD26/ dipeptidylpeptidase IV regulates CXCL12/stromal cell-derived factor-1 alphamediated chemotaxis of human cord blood CD34+ progenitor cells. J Immunol. 2002; 169(12): 7000–8.
105. Christopherson KW, Hangoc G, Mantel CR, et al.: Modulation of hematopoietic stem cell homing and engraftment by CD26. Science. 2004; 305(5686): 1000–3.
106. Campbell TB, Hangoc G, Liu Y, et al.: Inhibition of CD26 in human cord blood CD34+ cells enhances their engraftment of nonobese diabetic/severe combined immunodeficiency mice. Stem Cells Dev. 2007; 16(3): 347–54.
107. Farag SS, Nelson R, Cairo MS, et al.: High-dose sitagliptin for systemic inhibition of dipeptidylpeptidase-4 to enhance engraftment of single cord umbilical cord blood transplantation. Oncotarget. 2017; 8(66): 110350–111057.
108. Farag SS, Srivastava S, Messina-Graham S, et al.: In vivo DPP-4 inhibition to enhance engraftment of single-unit cord blood transplants in adults with hematological malignancies. Stem Cells Dev. 2013; 22(7): 1007–15.
109. Vélez de Mendizábal N, Strother RM, Farag SS, et al.: Modelling the sitagliptin effect on dipeptidyl peptidase-4 activity in adults with haematological malignancies after umbilical cord blood haematopoietic cell transplantation. Clin Pharmacokinet. 2014; 53(3): 247–259.
110. Broxmeyer HE, Hoggatt J, O'Leary HA, et al.: Dipeptidylpeptidase 4 negatively regulates colony-stimulating factor activity and stress hematopoiesis. Nat Med. 2012; 18(12): 1786–96.
111. Jia Z, Zhang Y, Ding G, et al.: Role of COX-2/mPGES-1/prostaglandin E2 cascade in kidney injury. Mediators Inflamm. 2015; 2015: 147894.
112. Nørregaard R, Kwon TH, Frøkiær J: Physiology and pathophysiology of cyclooxygenase-2 and prostaglandin E2 in the kidney. Kidney Res Clin Pract. 2015; 34: 194–200.
113. Dey I, Lejeune M, Chadee K: Prostaglandin E2 receptor distribution and function in the gastrointestinal tract. Br J Pharmacol. 2006; 149: 611–23.
114. Wang Y, Lai S, Tang J, et al.: Prostaglandin E2 promotes human CD34+ cells homing through EP2 and EP4 in vitro. Mol Med Rep. 2017; 16(1): 639–646.
115. Broxmeyer HE, Pelus LM: Inhibition of DPP4/CD26 and dmPGE2 treatment enhances engraftment of mouse bone marrow hematopoietic stem cells. Blood Cells Mol Dis. 2014; 53(1–2): 34–8.
116. Adams GB, Chabner KT, Alley IR, et al.: Stem cell engraftment at the endosteal niche is specified by the calcium-sensing receptor. Nature. 2006; 439(7076): 599–603.
117. Lam BS, Cunningham C, Adams GB: Pharmacologic modulation of the calciumsensing receptor enhances hematopoietic stem cell lodgment in the adult bone marrow. Blood. 2011; 117(4): 1167–75.
118. Hidalgo A, Frenette PS: Enforced fucosylation of neonatal CD34+ cells generates selectin ligands that enhance the initial interactions with microvessels but not homing to bone marrow. Blood. 2005; 105(2): 567–75.
119. Robinson SN, Simmons PJ, Thomas MW, et al.: Ex vivo fucosylation improves human cord blood engraftment in NOD-SCID IL-2Rγ(null) mice. Exp Hematol. 2012; 40: 445–56.
120. Wysoczynski M, Ratajczak J, Pedziwiatr D, et al.: Identification of heme oxygenase 1 (HO-1) as a novel negative regulator of mobilization of hematopoietic stem/progenitor cells. Stem Cell Rev Rep. 2015; 11(1): 110–8.
121. Adamiak M, Moore JB 4th, Zhao J, et al.: Downregulation of Heme Oxygenase 1 (HO-1) Activity in Hematopoietic Cells Enhances Their Engraftment After Transplantation. Cell Transplant. 2016; 25(7): 1265–76.
122. Tan CK, Wahli W: A trilogy of glucocorticoid receptor actions. Proc Natl Acad Sci U S A. 2016; 113(5): 1115–7.
123. Guo B, Huang X, Cooper S, et al.: Glucocorticoid hormone-induced chromatin remodeling enhances human hematopoietic stem cell homing and engraftment. Nat Med. 2017; 23(4): 424–428.
124. Peserico A, Simone C: Physical and functional HAT/HDAC interplay regulates protein acetylation balance. J Biomed Biotechnol. 2011; 2011: 371832.
125. Yang XJ, Seto E: HATs and HDACs: from structure, function and regulation to novel strategies for therapy and prevention. Oncogene. 2007; 26(37): 5310–8.
126. Huang X, Guo B, Liu S, et al.: Neutralizing negative epigenetic regulation by HDAC5 enhances human haematopoietic stem cell homing and engraftment. Nat Commun. 2018; 9(1): 2741.
127. Zhi Y, Lu H, Duan Y, et al.: Involvement of the nuclear factor-κB signaling pathway in the regulation of CXC chemokine receptor-4 expression in neuroblastoma cells induced by tumor necrosis factor-α. Int J Mol Med. 2015; 35(2): 349–57.
128. Huang B, Yang XD, Lamb A, et al.: Posttranslational modifications of NFkappaB: Another layer of regulation for NF-kappaB signaling pathway. Cell Signal. 2010; 22(9): 1282–90.
129. Forristal CE, Winkler IG, Nowlan B, et al.: Pharmacologic stabilization of HIF-1α increases hematopoietic stem cell quiescence in vivo and accelerates blood recovery after severe irradiation. Blood. 2013; 121(5): 759–69.
130. Galbraith MD, Allen MA, Bensard CL, et al.: HIF1A employs CDK8-mediator to stimulate RNAPII elongation in response to hypoxia. Cell. 2013; 153(6): 1327–39.
131. Speth JM, Hoggatt J, Singh P, et al.: Pharmacologic increase in HIF1α enhances hematopoietic stem and progenitor homing and engraftment. Blood. 2014; 123(2): 203–7.
132. Takubo K, Goda N, Yamada W, et al.: Regulation of the HIF-1alpha level is essential for hematopoietic stem cells. Cell Stem Cell. 2010; 7(3): 391–402.
133. Chen X, Han Y, Zhang B, et al.: Caffeic acid phenethyl ester promotes haematopoietic stem/progenitor cell homing and engraftment. Stem Cell Res Ther. 2017; 8(1): 255.
134. Broxmeyer HE: Long-Overdue Guidelines for the Cord Blood Banking Community. Stem Cells Transl Med. 2019; 8(4): 320–322.
Source: F1000Research